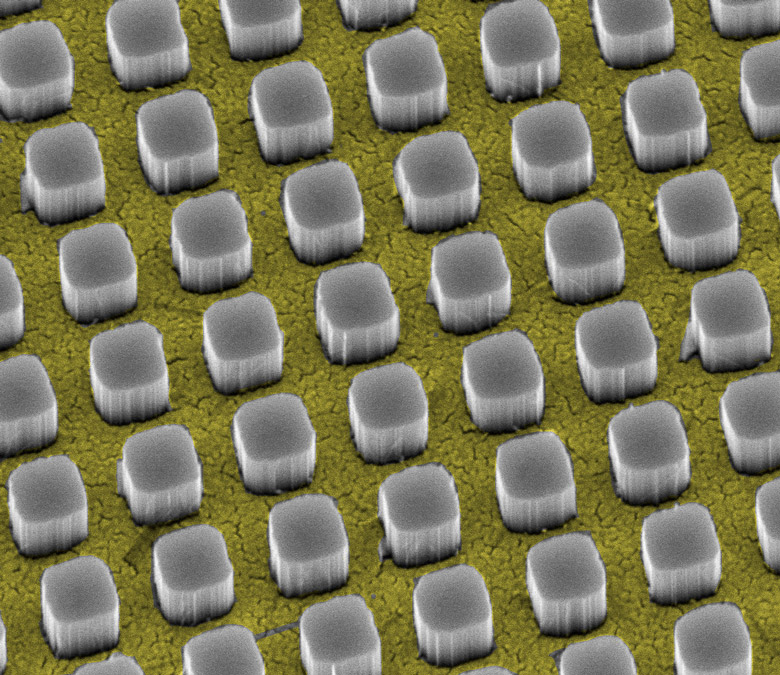
In recent years, there has been a lot of interest in coupling sound and light together. Admittedly, we've been doing this for a long time, but we've always been limited in terms of what we can do with how nature puts materials together. Now, with our ability to construct structures that are the right size, we can make devices that really dance to the tune that we give them.
This control has been demonstrated in a very cute way recently. Researchers have put together micro pillars that convert light into long-lasting, very high-frequency sound waves.
Nature leads the way
Nature, of course, allows sound and light to play together in different ways. For instance, if a gas absorbs light, it will heat up and expand, so flashing a light into a gas will generate a sound wave at the frequency of the flashing. One of the most sensitive techniques for measuring how materials absorb light makes use of this.
The Internet, surprisingly, relies on light-generating sound waves. To amplify the light traveling down an optical fiber, we put in a strong light field that is just slightly bluer in color than the light carrying the signal. The blue light excites sound waves in the fiber—these are sound waves with THz (1012Hz) frequencies, while audible sounds are in the kHz range. By generating sound waves, the blue light loses energy and turns redder. The photons from the signal act as a seed, encouraging the process that generates sound waves to produce red photons that amplify the signal, rather than randomly generating light at just any redder color.
The key requirement for this amplification process is that the material—glass in the case of optical fiber—has to support a sound wave at the desired frequency. That is not a given. The sound waves that can be supported by a material are controlled by the mass of the constituent atoms and the strength of the bond between the atoms.
We'd love to have more freedom in this area. With better control, for instance, we could create sound-lasers ("sasers"). That means we need to control how materials are put together at the scale of the acoustic wavelength. For audible frequencies, this is doable—think guitar strings, sounding boards, and organ pipes. But for sound frequencies in the GHz and THz range, the wavelength is less than a micron, which makes fabrication more difficult. The biggest problem is not the size, though. It is that, unlike light, sound waves really feel the material they are moving through. Any imperfection is keenly felt, and the sound waves are scattered and lost at these imperfections.
Pillars of sound and light
To get sound and light to play together as instructed, the researchers constructed little pillars that resonate with both sound and light. The pillars were made from layers of gallium arsenide and aluminum arsenide. These two materials grow very neatly on top of each other because their crystal structures are nearly identical. This creates an interface that has nearly no imperfections. But the materials are different enough that these interfaces reflect both sound and light quite strongly. This is unusual—many materials that are so similar have different optical properties, but their acoustic properties are similar enough that their acoustic reflections are very weak.
Now comes the cool bit: by growing repeating layers of the two materials with the right spacing, many sound and light reflections are created. For the sound and light wavelengths that match the layer spacing, the reflections all add up to make one very highly reflective mirror.
Two of these mirrors were grown with a small gap in between, creating a tiny space where both sound and light are reflected back and forth between the mirrors. This cavity builds up a very high intensity of acoustic and light waves. Or it would, if the light and sound didn't escape out the sides. To prevent that, the researchers etched most of the material away, leaving pillars just a few micrometers square. At these scales, the interface between the pillars and the air also strongly reflects light and sound and confines both to the gap between the mirrors.
Thunder in the pillars
To excite sound waves with light, the researchers blast the pillar with light pulses that are very short compared to the lifetime of the sound waves. Some of the light is absorbed by the material, setting up a short, sharp wave of material motion. The mirrors select just one sound wavelength and resonate that back and forth between them. The researchers showed that this sound wave persists for some 5 to 20 nanoseconds, depending on the acoustic frequency.
This is remarkable because these sound waves have frequencies in the 20 to 100 GHz range, which tend to be damped out in a very short time. This tells us that the two materials match nearly perfectly, and there are very few imperfections at the interfaces and in the bulk materials, and even the sidewalls are very smooth.
The researchers show that the sound-generating process is just like in the fiber amplifiers above. A small part of the bluer-colored light in the pulse is absorbed to create the sound wave. And, if you send in a second light pulse a short time later, the red part of the light pulse is absorbed to damp out the sound waves.
A tiny band with no bass
The researchers, of course, have their own ideas about what they plan to do with these devices. They're all about fundamental quantum mechanics: can we take such a pillar and put just a single phonon (the sound equivalent of a photon) of sound in the pillar? Can we entangle phonons and photons at such high frequencies?
What does all this mean? Imagine a mirror balanced on a spring (you actually need two mirrors to match what I describe below, but let's keep it simple). According to quantum mechanics, the mirror cannot sit still—there is a minimum amplitude of motion. Now, we send a single photon at the mirror. The photon reflects off the mirror, and two things may happen. The reflection of the photon can be enough to drive the mirror to vibrate more energetically. If that is the case, then the photon will have a slightly different color (it will be redder). If vibration doesn't result, then the photon stays the same color.
Until we measure either the motion of the mirror or the color of the photon, we have to treat the photon and the oscillating mirror as a single entity of a blue-photon + lazy-mirror, and red-photon + energetic-mirror. Measuring one will not just reveal but also set the state of the other. That is, if we discover that the mirror is moving more energetically, then we know the photon is red.
Measurement-wise, doing this with GHz sound waves is much easier than with kHz or MHz vibrating mirrors. But creating the conditions where the oscillator is in its quantum mechanical ground state is really difficult at high frequencies. So, this is the challenge that the researchers have taken on.
Personally, I am more excited about things like the operation of sasers at GHz and THz frequencies. I reckon clever people can find myriad applications of coherent sound. More to the point, think back to the light amplifiers that amplify fiber optic signal by generating waste sound waves. This development shows that we can actually tailor the sound waves supported by a material and hence, amplify light in ways that we couldn't before.
Admittedly, this is not yet at the frequency range where it is useful. What counts is the frequency and bandwidth of the sound wave. For fiber optic communications, the frequency of the sound wave is much larger, and the bandwidth is huge; this demonstration tops out at 100GHz, and the bandwidth is tiny (a few MHz compared to 100s of GHz). But I can imagine ways around these shortcomings. I can also imagine using the narrow bandwidth in various ways as well.
Physical Review Letters, 2017, DOI: 10.1103/PhysRevLett.118.263901 (About DOIs).
reader comments
19