Abstract
Specific expression patterns of microRNA (miRNA) molecules have been linked to cancer initiation, progression, and metastasis. The accumulating evidence for the role of oncogenic or tumor-suppressing miRNAs identified the need for nano-scaled platform that can help deliver nucleotides to modulate miRNAs. Here we report the synthesis of novel layered gadolinium hydroxychloride (LGdH) nanoparticles, a member of the layered double hydroxide (LDH) family, with physiochemical properties suitable for cell uptake and tracing via magnetic resonance (MR) imaging. As a proof of concept, we demonstrate the inhibition of mature miRNA-10b in metastatic breast cancer cell line using LGdH nanoparticle as a delivery platform. Through characterization analysis, we show that nanoparticles are easily and stably loaded with anti-miRNA oligonucleotides (AMO) and efficiently penetrate cell membranes. We demonstrate that AMOs delivered by LGdH nanoparticles remain functional by inducing changes in the expression of its downstream effector and by curbing the invasive properties. Furthermore, we demonstrate the traceability of LGdH nanoparticles via T1 weighted MR imaging. LGdH nanoparticles, which are biocompatible with cells in vitro, provide a promising multifunctional platform for microRNA therapeutics through their diagnostic, imaging, and therapeutic potentials.
Export citation and abstract BibTeX RIS
Introduction
MicroRNAs (miRNAs) are a class of small, non-coding RNAs that can regulate genes post-transcriptionally by mRNA cleavage or translational repression [1]. Recently, specific expression patterns or mutations of miRNAs were validated as causative factors in a wide variety of cancer malignancies [2, 3] including breast cancer [4, 5]. Although a majority miRNAs act as tumor suppressors, a subset of miRNAs display increased expression in cancer and act as oncogenes [6, 7]. Studies involving oncogenic miRNAs have demonstrated that anti-miRNA oligonucleotides (AMO) against the mature miRNA sequences can be used to effectively silence miRNAs in vivo [8–10]. Amongst many of the oncogenic miRNAs that have been identified, overexpression of miRNA-10b was shown to specifically promote early stage metastasis in breast cancer [9, 11, 12]. The use of AMOs against miR-10b in these studies was successful in curbing metastasis to distant organs, which rationalizes the effort to render a suitable delivery vehicle for AMO's clinical use in order to avoid possible immune response associated with naked oligonucleotides [13].
In this paper, we regulate the miR-10b expression in breast cancer cell lines using a novel delivery vehicle, layered gadolinium hydroxychloride (LGdH) (Gd2(OH)5(H2O)x)Cl nanoparticles. LGdH nanoparticles are structurally similar to a family of layered double hydroxides (LDHs) [14]. LGdH nanoparticle structure consists of positively-charged hydroxide layers of trivalent rare-earth gadolinium ions (Gd3+) with water molecules and exchangeable chloride ions in the interlayer space [15]. The hydrated anions in the interlayer space of LDHs are highly mobile, which allows for incorporation of desired anionic species by a direct ion exchange method of intercalation [16, 17]. The kinetics and mechanisms of ion exchange reaction between LDHs and various kinds of organic and inorganic anions have been extensively studied [16, 18–20]. The favorable electrostatic interactions between multivalent anionic biomolecules and cationic metal hydroxide layers owing to an ion exchange reaction has shown to increase stabilization energy [17] and lower thermodynamic energy [21]. As a result of this desirable property, LDHs need not be conjugated to gene therapy agents in order to deliver them. Many studies have demonstrated success in exploiting the strong anion exchange capability of LDHs by using it as a carrier of a range of gene therapy agents such as antisense oligonucleotide [22], plasmid DNA [23], and siRNA [24].
The family of LDH nanoparticles have several advantages over other vector formulations for the purposes of gene therapy. As a non-viral vector, LDH nanoparticles can minimize the side effects associated with immune response to the virus [25]. With the demonstrated biocompatibility and minimal cell toxicity [14], LDHs nanoparticles has been successfully used in a wide range of therapeutic applications [26, 27]. The shape of LDH nanoparticle can be controlled during the synthesis to target a specific sub-cellular compartment [28], which allows for delivery in a manner specific to the function of the loaded agent. The positive charge of the LDH nanoparticle surface is complementary with the negatively-charged cellular membrane [29], which encourage cellular uptake through clathrin-mediated endocytosis [30]. Furthermore, LDHs can effectively protect the agents from degradation during the uptake process [31, 32], whilst allowing for their recovery at the desired location [17] upon dissolution of LDHs in a low pH environment [14]. The intrinsic properties of LDHs make them suitable for cellular delivery applications.
In this paper we present the synthesis of a unique Gd3+-based LGdH nanoparticle and characterization of LGdH nanoparticles loaded with AMOs against miR-10b. As a material of high atomic number, rare-earth Gd3+-based nanoparticles are of great interest for their magnetic resonance(MR) imaging contrast [14, 33] and radiation-therapy enhacing capabilities [34, 35]. In this paper, we explore the application of LGdH nanoparticles by examining the delivery efficiencies and testing the functionality of LGdH nanoparticles loaded with AMO in vitro, and validating its clinical applicability via MR imaging.
Material and methods
Preparation and characterization of LGdH and AMO-loaded LGdH nanoparticles
LGdH nanoparticles were synthesized using a previously reported procedure with minor modifications [14]. Briefly, 5.75 ml of 1.5 M NaOH was added to 20 ml of 0.125 M GdCl3·6H2O with vigorous stirring at room temperature to adjust the final pH to 6.5. The precipitate was heated at 80 °C for 2 h with vigorous stirring. The slurry of LGdH was centrifuged at 6000 rpm for 10 min and the supernatant was decanted. Nanoparticle pellet was washed thoroughly with deionized water and dispersed with a sonic dismembrator (Model 705, Fisher Scientific). The sizes of LGdH nanoparticles were determined by using the ellipse tool in the ImageJ program (Ver 1.48d, National Institute of Health).
A single-stranded miR-10b inhibitor, miRCURY LNA miRNA Inhibitor with the sequence of 5'-ACAAATTCGGTTCTACAGGGT-3' was used as AMO (Exiqon, Woburn, MA, USA). The equivalent oligodeoxynucleotide-based sequences in untagged and 5' Alexa Fluor 488-modified forms were used for characterization, confocal microscopy, and flow cytometry studies (Integrated DNA technologies). AMOs were loaded to LGdH nanoparticles via ion exchange. AMO and nanoparticle were mixed in deionized water (pH 7.0) at 1:1 mass ratio and vortexed immediately at the highest setting. The mixture was then shaken on a roller for 30 min, centrifuged, and resuspended in saline at room temperature prior to use.
The powdered LGdH and AMO-LGdH nanoparticle samples for x-ray diffraction (XRD) and Fourier transform infrared spectroscopy (FTIR) were obtained by vacuum drying the sample at room temperature. The XRD analysis was conducted on a Rigaku Ultima IV Powder XRD system run at 38 kV and 38 mA using a x-ray generator with a cobalt tube. The search and match was done with JADE software (version 6.1.7601, Materials Data). The FTIR spectrophotometry data (Nicolet 8700, Thermo Scientific) was analyzed via OMNIC software (version 6.2, Thermo Electron Corporation). Zeta potential measurements were recorded at 25 °C in triplicates (Zetasizer Nano-ZS, Malvern Instruments). The average of zeta potential measurements is reported ± standard error of mean.
AMO loading efficiency
For the determination of AMO loading and entrapment efficiencies, amount of AMO in the supernatant was assessed via Beckman UV spectrophotometer. Calculations were done according to the following [36]:
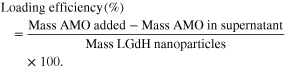
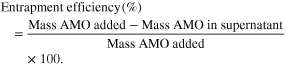
Experiment was conducted in triplicates.
Cell culture
Human breast cancer cell lines MDA-MB-231 and MCF7 were obtained from American Type Culture Collection (ATCC) and cultured in Dulbecco's Modified Eagle Medium (Gibco) supplemented with 1% antibiotic-antimycotic (Invitrogen), 5 μg ml−1 plasmocin prophylactic (Invivogen), and 10% fetal bovine serum (FBS; Gibco). Cells were kept in polystyrene flasks (Corning) in incubator with 5% CO2 at 37 °C.
Cell uptake
For preparation for TEM, MDA-MB-231 cells were seeded onto sterile plastic cover slips in a six-well plate 24 h before the treatment. On the day of the treatment, cells were treated with 200 μg ml−1 LGdH nanoparticle and incubated for 1, 6, 24, or 48 h. The cells on cover slips were washed twice with PBS, and then fixed with 2% paraformaldehyde and 2.5% glutaraldehyde. The cells were then dehydrated with an ethanol and block stained with 1% uranyl acetate, infiltrated by Spurrs' resin, and polymerized in 65°–70 °C oven. Thin sections of 100 nm were cut with ultramicrotome (Leica EM UC6) and coated on a 400 mesh copper grid. The sections were stained with 2% Uranyl acetate and 1% lead citrate.
Transmission electron microscopy
The images were taken using JEM 2100 transmission electron microscope at 120 kV (JEOL).
In vitro cell toxicity
Viability of cells treated with 20, 50, 100, or 200 μg ml−1 concentrations of LGdH nanoparticles or AMO-loaded LGdH nanoparticles was determined using the AlamarBlue Cell Viability Reagent (Invitrogen) as per manufacturer's instructions. Briefly, MDA-MB-231 cells were seeded at 4 × 103 cells per well on a 96-well assay plate (Corning) and allowed to attach for 24 h. On the next day, cells were treated and incubated for another 48 h. After the addition of AlamarBlue reagent, cells were incubated for 4 h. Absorbance was monitored at 590 nm using Optima 96-well plate reader (BMG LABTECH). Experiment was conducted in quadruplicates.
Cell transfections
MDA-MB-231 cells were seeded onto six-well culture plates (105 cells per well) and allowed to attach for 24 h. Media in all the wells were replaced with DMEM supplemented with 10% FBS without antibiotics prior to transfections. AMO-LGdH nanoparticle complexes were prepared at 18 μg: 18 μg weight ratios, and were added to each well to give a final LGdH concentration of 9 μg mL−1. Transfections using Lipofectamine RNAiMAX (Life Technologies) were performed following the manufacturer's protocol. About one-half of AMO in AMO-LGdH nanoparticles was used for AMO-lipofectamine and AMO-only groups to compensate for the loading efficiencies. Cell transfections were complete in 24 h.
Migration and invasion assay
Cells were starved for 24 h in serum-free DMEM medium prior to the assay. Both migration (8 μm Mililcell cell culture inserts, Merck Millipore) and invasion assays (8 μm QCM™ High Sensitivity Non-cross-linked Collagen Invasion Assay, Merck Millipore) were conducted as per manufacturer's instructions. Briefly, after plating cells in inserts, cells were incubated for 6 h for the migration assay and 72 h for an invasion assay. Media supplemented with 10% FBS and 20% FBS was used as a chemo-attractant for the migration and invasion assays, respectively. For the migration assay, inserts were washed twice with PBS, fixed with 4% paraformaldehyde at room temperature, permeabilized with 100% methanol, and stained with crystal violet. For the invasion assay, staining reagent in the kit was used as per manufacturer's instructions. Cotton swabs were used to scrape off non-migrated cells from the top membrane prior to imaging under Olympus IX70 microscope. One center field views was taken per well using Olympus Plan 10X/0.25PhI or UPlanFl 20x/0.5 lenses, Zeiss Axiocam MRc camera, and AxioVision software (Version 4.4.0.0, Carl Zeiss). Both assays were conducted in triplicates. The numbers of migrated cells were counted with Metamorph software (64-bit Version 7.8.4.0; Molecular Devices).
Confocal imaging
MDA-MB-231 cells were seeded onto glass cover slips (Corning) 24 h prior to treatment in a six-well plate. The day after transfection, the media was removed and the wells were washed three times with warm phosphate buffered saline (PBS; Gibco, USA). Cells were fixed in warm 4% paraformaldehyde (Sigma Aldrich) in PBS for 20 min The cover slips were washed twice in PBS, and then mounted on glass slides using a mounting medium with DAPI (Life Technologies). Images were acquired on a Zeiss LSM-710 laser scanning microscope (Carl Zeiss) with 40 × 1.3 Oil DIC M27 using ZEN 2011 software (black edition 64-bit, version 7.0.4.0, Carl Zeiss). All treatment groups were prepared in triplicates.
Flow cytometry
Flow Cytometry was conducted to determine the cell uptake of 5' Alexa Fluor 488-modified AMO-LGdH nanoparticles. The day after transfection, cells were trypsinized and centrifuged at 200 g for 5 mins. After removal of the supernatant, cells were washed three times with PBS. Cells were analyzed by a FACSCalibur flow cytometer (BD Biosciences, San Jose, CA) with a 15 mW air-cool argon-ion laser at an excitation frequency of 488 nm. Fluorescence signals were detected using a band-pass filter 530/30 nm. The BD FACSCalibur™ flow cytometer was automatically set up and compensated with BD Calibrite™ beads used with FACSComp™ software (BD Biosciences). Experiments were conducted in triplicate.
Western blotting
Cells were washed twice with PBS (Gibco) and whole cell lysates were harvested in lysis buffer (150 mM sodium chloride, 1% Triton X-100, 50 nM Tris, pH 8.0) using a cell scraper (Corning). Protease inhibitor cocktail (Sigma Aldrich) was added to the collection tube. Proteins were resolved by Mini-Protean TGX 4–20% gel (Biorad), transferred to PVDF membrane (Biorad), blocked in 5% skim milk in TBST, and blotted with antibodies for HOXD10 (1:1500, Abcam) and α-tubulin (1:2000, Abcam), and secondary antibody conjugated to HRP (1:2000, Abcam). Clarity Western ECL Substrate (Biorad) was used for film-based imaging.
MR imaging
Magnetic strength measurements were made with vibrating sample magnetometer (VSM 7400, Lakeshore Cryotronics) at room temperature. 3.0T Philips MRI using spin-echo sequence was used to acquire T1-weighted image (TE, 8 ms; TR, 800 ms).
Statistical analysis
Statistical significance for migration and invasion were determined by a one-way analysis of variance (ANOVA) followed by Dunnett's multiple comparison test with Graphpad Prism software (Prism 5 for Windows, Version 5.04, GraphPad Software). Statistical significance for cell toxicity was determined by a two-way analysis of variance (ANOVA) followed by Bonferroni multiple comparison tests. P values of <0.05 were considered to be statistically significant for all tests. Graphs produced by Graphpad Prism software are presented as means with error bars representing standard error of mean.
Results
Characterization of AMO-loaded LGdH nanoparticles
The protocol described in Materials and Methods produced homogenous and stable aqueous suspension of oval LGdH nanoparticles (figure 1(A)). The LGdH nanoparticles had an average major and minor diameters of 146 nm and 90 nm, respectively, within the range for effective endocytosis [29]. We tested several AMO to LGdH nanoparticle mass ratios to assess the loading and entrapment efficiencies. The loading efficiency was highest for the highest AMO to LGdH nanoparticle ratio tested (6:1), whereas the entrapment efficiency was highest for the lowest AMO to LGdH nanoparticle ratio tested (0.5:1) (figure 1(C)). At 1:1 AMO to LGdH nanoparticle mass ratio, the loading and entrapment efficiencies were 41.8% and 89.5%, respectively, and this ratio was selected for all the subsequent experiments. The apparent size and shape of LGdH nanoparticles did not change after the addition of AMO (AMO-LGdH; figure 1(B)), which indicated effective intercalation of AMOs into the interlayer spaces of LGdH nanoparticles [24]. Intercalation activity of AMOs into LGdH nanoparticles was further confirmed by the absence of agglomeration, which has reported to occur for LDHs subjected to suboptimal intercalation activity [37]. In line with previous observations for LDHs loaded with anionic biomolecules [17, 22, 24], a decrease in positive surface charge was observed for our AMO-loaded LGdH nanoparticles. The LGdH nanoparticles loaded with AMOs as measured by zeta potential had more neutral electrical characteristic in an aqueous solution with zeta potentials of +14.6 ± 0.1 mV compared to +45.6 ± 0.4 mV for pristine LGdH nanoparticles (figure 2).
Figure 1. TEM images of (A) pristine LGdH nanoparticles and (B) the same LGdH nanoparticles associated with AMO. The morphology of LGdH nanoparticles remains the same upon association with AMOs. (C) AMO loading and entrapment percentages for a range of AMO to LGdH nanoparticle mass ratio preparations.
Download figure:
Standard image High-resolution imageFigure 2. Zeta potential distributions of AMO and AMO-LGdH nanoparticles. The decrease in zeta potential reflects extensive AMO intercalation into interlayers of LGdH nanoparticles.
Download figure:
Standard image High-resolution imageFurther characterization was conducted to confirm the association of AMOs with LGdH nanoparticles. The FTIR spectrum of the both samples showed absorption peaks that are characteristic of rare earth metal-based hydroxycarbonate nanoparticles with the vibration of the OH-group at 3525 cm−1 and 3497 cm−1 (figure 3) [38]. From the analysis of deconvolved FTIR spectra of the AMO-loaded LGdH nanoparticles, we were able to identify peaks that were absent in pure LGdH spectra that could specifically be attributed to nucleic acids (data not displayed). The bands at 1052 cm−1 and 1223 cm−1 could be assigned to C-O stretching vibration of deoxyribose or ribose residue and symmetric stretching vibration of PO2− group, respectively [39]. The characteristic bands were also present for in-plane base vibrations of thymine (1695 cm−1), guanine (1588 cm−1), and cytosine (1529 cm−1), as well as ring vibrations of guanine and adenine (1482.5 cm−1 and 1308 cm−1) (figure 3) [40]. The FTIR result confirmed that AMO-LGdH nanoparticles consist of oligonucleotides and LGdH nanoparticles, and that the intrinsic structure of LGdH nanoparticles remains intact even after intercalation of AMOs into interlayer spaces.
Figure 3. FTIR spectra of LGdH and AMO-LGdH nanoparticles. FTIR spectra of AMO-LGdH nanoparticles display additional absorption bands characteristic of oligonucleotides.
Download figure:
Standard image High-resolution imageIn order to further confirm the association between LGdH nanoparticles and AMOs, we employed XRD to compare the crystal structures (figure 4). The XRD peaks for both samples were ascribed to hydrotalcite (JCPDS card 22–700), the magnesium and aluminum hydroxycarbonate that has a structure of layered double hydroxide. The basal spacing of pristine LGdH nanoparticles was calculated to be 0.893 nm, and this spacing did not expand with the addition of AMOs. The structural information based on XRD patterns indicated that AMO-LGdH nanoparticles retained the crystal structure of the pristine LGdH nanoparticles, as the only difference in the XRD patterns was the overall decrease in the diffraction intensity. This suggested that that AMOs were intercalated into the interlayer spaces without disturbing the basal spacing of pristine LGdH nanoparticles. The association of AMOs with LGdH nanoparticles without expansion was an expected outcome as the dimensions of a single-stranded AMO molecule does not necessitate an expansion of LGdH nanoparticles' interlayer space in order to intercalate [41, 42]. Overall, our characterization data demonstrated that AMOs associate with LGdH nanoparticles by intercalating within the interlayer regions of LGdH nanoparticles. Our data, together with the knowledge on small nucleic acids intercalated into interlayer spaces of LDHs [17], confirmed successful intercalation through electrostatic interactions between the negatively charged phosphate groups of the AMOs with the positively charged gadolinium hydroxide layers of LGdH nanoparticles.
Figure 4. Powder x-ray diffraction patterns of LGdH and AMO-LGdH nanoparticles. Decrease in diffraction intensity for AMO-LGdH nanoparticles indicate AMO-induced distortions of basal layers.
Download figure:
Standard image High-resolution imageBiocompatibility of LGdH nanoparticles
The cell toxicity of LGdH and AMO-LGdH nanoparticles was tested for a range of LGdH nanoparticle concentrations (or equivalent LGdH nanoparticle concentrations for AMO-LGdH group) in MDA-MB-231 cells (figure 5). The maximum concentration and the treatment duration tested were ten times and two times greater, respectively, compared to the parameters used for all the subsequent studies. As previously reported for the LDH nanoparticles [26, 27], LGdH nanoparticles did not cause significant cell toxicity for concentrations up to 200 μg ml. In addition, viability of cells treated with AMO-LGdH nanoparticles were not significantly different from cells treated with pristine LGdH nanoparticles for all the concentrations tested. This result confirmed that LGdH nanoparticles are biocompatible and that miR-10b antagonism alone does not affect the viability of the metastatic cell line [12].
Figure 5. Alamar blue cell viability data of MDA-MB-231 cells treated with specified concentrations of LGdH or AMO-LGdH nanoparticles for 48 h.
Download figure:
Standard image High-resolution imageUptake of LGdH nanoparticles by breast cancer cells
To characterize LGdH nanoparticles as a delivery platform, we tested whether cancer cells can readily take up LGdH nanoparticles at the specified time points. Using TEM, we observed cytosolic localization of nanoparticles in MCF7 cells at both early and later time points. At one-hour time point, LGdH nanoparticles were primarily at the membrane interface with minimal cytosolic localizations (figure 6(A)). After six hours of incubation with LGdH nanoparticles, considerable amount of LGdH nanoparticles were internalized in the endosomal compartments (figure 6(B)). After a day of incubation, some LGdH nanoparticles were degraded inside the endosomal compartments, as evidenced by the decrease in densities of LGdH nanoparticles in the endosomes compared to extracellular nanoparticles (figure 6(C)). The empty endosomal structures as well as LGdH nanoparticle-containing endosomes were visible on a second day (figure 6(D)).
Figure 6. TEM images showing intracellular localization of LGdH nanoparticles in MCF7 cells at (A)1 h, (B) 6 h, (C) 24 h, and (D) 48 h post-treatment.
Download figure:
Standard image High-resolution imageLGdH nanoparticle mediated delivery of miR-10b AMO
The internalization of 5'-AlexaFluor488-tagged AMO-LGdH nanoparticles was visualized via confocal imaging and quantified via flow cytometry. Punctate localizations were observed once AMO-LGdH nanoparticles were internalized into MDA-MB-231 cells, confirming the endosomal compartment localization as observed under TEM (figure 7). Similar punctuate appearance was seen with AlexaFluor-488 tagged AMO transfected via Lipofectamine (AMO-Lipo) method. Fluorescence signal was detected in almost all cells for AMO-LGdH and AMO-Lipo groups, whereas no apparent signal was detected in cells treated with AlexaFluor488-AMOs without a vehicle (AMO-Only). Flow cytometry demonstrated that transfection was efficient for both the AMO-Lipo and AMO-LGdH treatment groups with more than 99% of cells treated being positive with fluorescence signal (figure 8). This transfection efficiency is in line with a previously reported value for short oligodeoxynucleotide delivered by LDH nanoparticle [24]. Despite the similar efficiencies in transfection, AMO-LGdH group had a broader distribution compared to the AMO-Lipo group indicating a greater variability in the number of AMO-LGdH taken up per cell. The absence of fluorescence signal in AMO-only group confirmed the results of our confocal imaging results.
Figure 7. Confocal fluorescence microscopic images of MDA-MB-231 cells treated with AlexaFluor488-tagged AMOs using lipofectamine (top; AMO-Lipo), LGdH nanoparticles (middle; AMO-LGdH), or no agent (bottom; AMO only).
Download figure:
Standard image High-resolution imageFigure 8. Flow cytometry histogram of AlexaFluor488-tagged AMO uptake using lipofectamine, LGdH nanoparticles, or no transfection and untransfected control. Percentage values are representative of experiment conducted in triplicates.
Download figure:
Standard image High-resolution imageDelivery of functional AMO by LGdH nanoparticles
It was previously reported that LDH nanoparticles carry biological molecules in the interlayer spaces for subsequent release in acidic buffer below pH 2 or in buffer of sodium chloride concentrations similar to that of bodily fluid without structural compromises to the cargo molecules [43]. Although our characterization studies revealed that many AMOs are likely to be absorbed onto the surfaces than intercalated between the layers, we tested whether LGdH nanoparticles can deliver and confer protection for the AMOs in vitro. For this, we used an AMO against miR-10b, a miRNA whose expression is known to correlate with metastatic potential of the cancer cells [12, 44]. We specifically tested changes in the migration and invasion properties, which are two separate steps in early metastasis (figure 9) [45]. We observed that AMO against miR-10b primarily affected invasive properties of MDA-MB-231 cells, with no significant decrease in the motility of MDA-MB-231 cells. AMO against miR-10b significantly reduced the invasive properties of the cell when transfected by LGdH nanoparticles. No significant difference was observed between the AMO-Lipo and AMO-LGdH nanoparticle groups. Our result is in line with previously reported result in which miR-10b inhibition specifically affected invasion but not migration in MDA-MB-231 cells [12].
Figure 9. Migration and invasion assay data for MDA-MB-231 treated with AMOs using lipofectamine, LGdH nanoparticles, or no transfection agent. Data for migration and invasion assays represent experiments conducted in triplicates.
Download figure:
Standard image High-resolution imageWe further tested the integrity of AMOs delivered by LGdH nanoparticles by checking the protein expression of miR-10b downstream effector (figure 10). Although the role of miR-10b has been implicated in numerous molecular pathways, the pathway leading to the metastatic program in breast cancer has been shown to include a pro-metastatic transcription factor Twist [12]. Twist directly induces the expression of miR-10b, which in turn indirectly targets homeobox D10 (HOXD1O) mRNA and inhibits its translation [12]. Inhibition of HOXD10 expression results in the increase of Ras homolog gene family, member C (RHOC) protein activity, a positive regulator of the metastic program in cells [46, 47]. With LGdH nanoparticles, we successfully delivered AMO against miR-10b and obtained HOXD10 expression similar to that for AMO-Lipo group (figure 10). HOXD10 expression was absent in the AMO-only group. This indicated that LGdH nanoparticles deliver functional AMOs in vitro by conferring some degree of protection in addition to promoting efficient cancer cell uptake.
Figure 10. Western blot data for whole cell lysates of MDA-MB-231 treated with AMO using lipofectamine, LGdH nanoparticles, or no transfection agent.
Download figure:
Standard image High-resolution imageMR imaging characteristics
To confirm that LGdH nanoparticles can be tracked with MR imaging, we obtained magnetization graph for LGdH nanoparticles (figure 11(A)) and assessed whether an effective T1-weighted MRI image could be taken in 3.0 T clinical MR scanner (figure 11(B)). The LGdH nanoparticles exhibited paramagnetic property as demonstrated by a linear relationship between magnetization and applied field. Concentration-dependent T1 signal intensity was seen for LGdH nanoparticles suspended in deionized water for concentration up to 5 mM, with no further contrast enhancement occurring between 5 mM and 10 mM. The MR imaging result demonstrated that the gadolinium-containing LGdH nanoparticles exhibit good T1 relaxivity and can be an effective T1 MRI contrast agent at concentrations below 5 mM.
Figure 11. The magnetic properties of LGdH nanoparticles in aqueous suspension. The LGdH nanoparticle (A) magnetization graph shows linear relationship between magnetization and applied field with accuracy better than 1% of reading and (B) T1-weighted MR imaging shows contrast enhancement in in vitro.
Download figure:
Standard image High-resolution imageDiscussion
In breast cancer, the roles of miRNAs are gaining emphasis in cancer initiation, progression, and metastasis [48–52]. Many links have been made between the expression signatures of miRNAs with clinicopathological characteristics and patient survival [5, 12, 50, 53, 54]. Abnormal expression profiles of miRNAs in cancers indicate that modulation of miRNA level via inhibitors or mimics may be useful for therapeutic purposes; however, safe and efficient delivery platform for miRNA modulators has yet to be reported [55]. In this paper, we studied LGdH nanoparticles as a novel AMO delivery platform to showcase LGdH nanoparticles as a potential multifunctional oligonucleotide carrier and further miRNAs as the potential cancer therapy target.
We demonstrated that LGdH nanoparticles successfully protect AMOs from premature degradation, confer good intracellular penetration via endocytosis, and allow intracellular drug release, while being biocompatible and traceable via MR imaging. The decrease in diffraction intensity according to XRD curve indicated that AMOs were exchanged with interlayer chloride ions while causing basal plane distortions [21]. Furthermore, the absence of interlayer space expansion indicated that AMOs were loaded parallel to the basal plane of hydroxide layers. The fact that AMOs were protected during the delivery into cells and able to inhibit miR-10b at the protein and functional levels further confirmed successful intercalation of AMOs. The observed decrease in positive zeta potential value for AMO-LGdH nanoparticles is a common phenomenon for anionic biomolecules loaded into the interlayer spaces of LDH nanoparticles [17, 22, 24], as the kinetics dictates that a proportion of negatively charged biomolecules need to be adsorbed onto the LDH surfaces prior to intercalation [18]. Nonetheless, the surface charge of AMO-LGdH nanoparticles remained sufficiently positive for efficient cellular uptake even at a relatively high mass ratio of AMOs used for this study [56].
Good intracellular penetration of AMOs was an expected outcome owing to efficient charge neutralization of oligonucleotides' negatively charged phosphate backbone by positively charged surface of LDH nanoparticles [17, 22]. This charge neutralization is advantageous for potential in vivo applications since it allows for greater stability in circulation while maintaining favorable interaction with the cell membrane [22, 29]. Our data showed that LGdH nanoparticles permit more efficient delivery and cellular effect compared to the naked AMOs, as less than half of the previously reported concentrations could induce functional changes in vitro [9, 57]. The delivery of functional AMOs against miR-10b reduced invasive activity through dis-inhibition of its downstream target HOXD10. By enhancement of the HOXD10 expression, subsequent invasive activities could be suppressed. In this paper, the loading of AMOs took only 30 min without complicated reaction steps, indicating that LGdH nanoparticles can be easily adopted as a delivery platform for other targets; other small-fragment oligonucleotides as well as small negatively charged molecules may be similarly loaded for delivery. MiR-10b is only one of the many regulated by Twist as a part of metastatic program [9]; hence, treating cancer cells with AMOs against multiple downstream targets of Twist, or miRNAs implicated in signatures, may be efficacious for the purposes of cancer therapeutics and should be explored further.
Breast MR imaging is currently indicated for women with greater lifetime risk for breast cancer as it is one of the most sensitive method available to provide a soft tissue contrast [58]. Using the conventional Gadolinium-based contrast agent, high quality images can be obtained for the primary tumor; however, it lacks the ability to accurately follow metastasis, a spread from the primary tumor to a secondary site, non-adjacent organs, or tissues [59]. This shortcoming is due to the rapid washout of the agent owing to its low molecular weight [58].
The benefit of using LGdH nanoparticles lies in the potential to improve both the diagnostic and therapeutic performances via exploiting their MR traceability. As a high-molecular weight gadolinium-based molecule, LGdH nanoparticles have the capacity to accumulate preferentially in tumor regions by taking advantage of abnormal openings in vessels, absence or lack of functional lymphatics, and angiogenic activities [60]. These properties, collectively called enhanced permeability and retention (EPR) effect [61], allow for passive accumulation of macromolecules in vivo as long as the plasma concentration remains high [62]. The EPR effect was demonstrated by the ultrasmall particles of iron oxide mediated improvement of diagnostic performance for lymph-node metastasis in clinics [63]. Furthermore, the fact that nanoparticles continue to circulate in the bloodstream and accumulate in tumors over the period of days to weeks permit less frequent intravenous administration compared to the conventional contrast agents [62]. The added benefit of using nanoparticles is the ability to load the therapeutic agents within them, allowing for tracing of the nanoparticles with real-time monitoring via T1-MR imaging to ensure that the target has been reached. Recently, gadolinium oxide nanoparticle encapsulated in phospholipid micelle demonstrated success as a multifunctional platform in vivo with good MR imaging, gene delivery, and cell uptake characteristics [33]. Although our in vitro experiments were conducted using the lower end of concentrations used for T1-MR imaging, our cell toxicity data as well as previous characterization of LGdH nanoparticles [14] demonstrate that the concentration can be increased to clinically relevant concentrations for greater contrast. Specific targeting of LDHs has not been explored in depth as a result of difficulties owing to its ionic nature and ways in which functionalization negate its inherent advantages [64, 65]. Research in this area may offer further benefits for gene delivery, by enhancing cellular uptake of nanoparticles that have passively accumulated within the tumor. Nonetheless, the combined diagnostic and therapeutic potential in addition to the imaging properties of LGdH nanoparticle make it a true multifunctional delivery platform.
Conclusion
We undertook the study with miRNA inhibitor with a novel delivery vehicle for their potential clinical use. The results in this paper indicate LGdH nanoparticles as a miRNA therapy delivery platform with good cellular uptake profile leading to inhibition of miR-10b function in vitro. The narrow size distribution, stability in hydrophilic environment, and traceability with T1-MR imaging suggest LGdH nanoparticles as a non-toxic imaging platform. LGdH nanoparticles have the potential for both soft tumor detection and treatment with miRNA modulators or other small-molecule chemotherapeutic agents. Given these combined multifunctional potentials, long-term in vivo toxicity, pharmacokinetic, and biodistribution studies are warranted for LGdH nanoparticles to further its clinical relevance.
Acknowledgements
We are grateful to Canadian Breast Cancer Foundation and Alberta Health Services for generously supporting this work. We thank National Institute for Nanotechnology, and Flow Cytometry Lab in Department of Experimental Oncology at the Cross Cancer Institute for the technical support, and Miss Natalie Estabrooks for her help in formatting and submission of the manuscript.